Research topics
Below, we explain and summarize the specific research projects pursued within our section: after a short general introduction into primordial structure formation, we present the first three projects, which are grouped along the cosmic timeline. The next five items represent more physics-based investigations, which are expected to have an important impact to cosmological structure formation. Finally we describe our CosmoSim database.
General introduction to the formation of the first cosmological structures
In the early Universe, quantum fluctuations were inflated to macroscopic size according to the theory of cosmic inflation. These fluctuations were then present in the density fields of dark matter, the ionized gas, and the photon field. Once these fluctuations entered the sound horizon of the cosmic plasma, the gravitational attraction in over-dense regions was balanced by the radiation pressure of photons, which led to propagating acoustic oscillations. The Universe continued to expand and to cool adiabatically. When the characteristic temperature of 3000 K was reached, protons and electrons were able to recombine to neutral hydrogen gas and the Universe became transparent to photons, which freely streamed away from the regions of last scattering. As a result, radiation pressure ceased to act as restoring force and the acoustic oscillations did not get excited any more. The initial fluctuations in the density of the released photons combined with gravitational redshift and Doppler effects get imprinted as fluctuations in the cosmic microwave background with a characteristic amplitude of one part in 100,000.
Since dark matter does not interact (electromagnetically) with photons, it had time to form tiny potential wells through gravitational interactions before recombination. Once set free from oscillations, the almost neutral primordial gas streams into those wells. The fluctuations continue to grow and accumulate more mass until they become non-linear. As they collapse in on themselves, these primordial dark matter halos decouple from the general Hubble expansion of the Universe. When the continuously infalling gas impacts on to the dense halo gas, shock waves form. These shock waves decelerate the cold gas, thereby converting the kinetic into thermal heat energy at the (low) characteristic virial temperature of the halos. The time gas takes to cool in these mini-halos (the “cooling time”) is sufficiently short so that gas quickly cools and collapses to very high densities; cold, high density gas is what is needed to form the first stars in the Universe. On-going star formation leads to the emergence of first galaxies.
Overview of projects
1. First stars, galaxies and AGN, and cosmic reionization
2. Exploring the impact of cosmic rays on galaxy formation
3. Formation and evolution of galaxy clusters
4. The interstellar medium and the physics of galactic outflows
5. Astroplasma physics: microscopic transport of cosmic rays and heat in galaxies and clusters
6. Physics and cosmology of blazar heating
7. Testing the Lambda-Cold Dark Matter cosmological model
8. Mapping particle physics to cosmology with an effective theory of structure formation - ETHOS
9. Cosmological simulations database - CosmoSim.
1. First stars, galaxies and AGN, and cosmic reionization
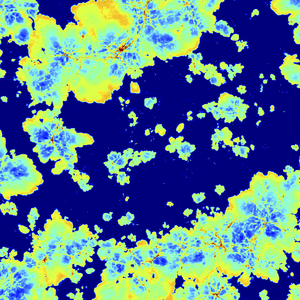
Temperature distribution in the intergalactic medium during patchy cosmic reionization
Credit: AIP/E. PuchweinThe formation of first stars, galaxies, and active galactic nuclei (AGN) profoundly affects their environment, including the low density intergalactic gas around them. The resonant scattering of energetic radiation emitted by the first young massive stars on surrounding gas couples the spin temperature of neutral hydrogen, which controls the occupation of its hyperfine states, to its kinetic temperature. This makes intergalactic gas and the cosmic structures it follows visible against the CMB, first in absorption and later in emission in the 21cm hyperfine structure line of neutral hydrogen. Large current and upcoming radio observatories and experiments such as HERA and SKA are aiming to measure this signal to sensitively probe star formation and cosmic structure growth in this early epoch. In our section, we perform cosmological hydrodynamical and radiative transfer simulations to investigate these processes in detail and to provide a solid theoretical basis for current and upcoming observational efforts.
Ionizing photons emitted from first galaxy populations also drive ionization fronts through the intergalactic medium, transforming it from a neutral gas into a highly ionized plasma. This process is called cosmic reionization. At the same time the energy deposited by the absorbed radiation heats the intergalactic medium by orders of magnitude. The heating can evaporate gas from low mass objects and/or prevent further gas infall, thereby suppressing galaxy formation in small halos. To explore when and how this reionization process happened and what the properties of the responsible ionizing galaxy and quasar populations were, we compare our simulations to Lyman-alpha absorption studies of the intergalactic medium. This includes comparisons of our simulations to absorption in the spectra of observed highest redshift quasars, as well as to the measured visibility of distant Lyman-alpha emitting galaxies. Constraining cosmic reionization in this way provides new insights into the high redshift galaxy populations that drive it, while at the same time mitigating degeneracies between the timing of reionization and measurements of other cosmological parameters. Furthermore, we investigate how the heating provided by cosmic reionization alters galaxy formation.
Lead investigators: Ewald Puchwein, Nele Stachlys (all AIP), James Bolton (Nottingham), Martin Haehnelt (Cambridge), Laura Keating (Edinburgh), Rahul Kannan (York U), Aaron Smith (U of Texas, Dallas)
2. Exploring the impact of cosmic rays on galaxy formation
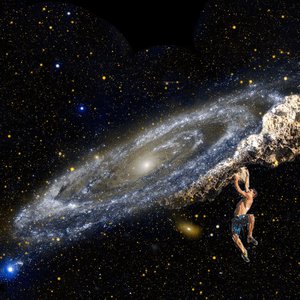
Cragsman logo: A composite image of a cragsman challenged by our neighboring Andromeda galaxy
Credit: NASA/JPL-Caltech/PfrommerOnce the dark matter halos reach the size of dwarf galaxies, gravitational attraction becomes stronger than the gas pressure. As a result, the gas collapses, becomes denser and radiatively cools in order to form a rotating gas disk in the halo center. Stars start to form in this disk and we witness the birth of a disk galaxy. Since the cooling time of the gas is considerably shorter than age of the galaxies, eventually all gas should have cooled, collapsed and formed stars. But this does not happen: while only 20% of the available gas is converted to stars in Milky Way-sized galaxies, even less (about 1% of the gas) ends up in stars in dwarf galaxies.
Understanding the physics behind this conundrum of galaxy formation is arguably among the greatest problems in modern astrophysics. Recent cosmological simulations have demonstrated that “feedback" by star formation, supernovae and accreting super-massive black holes (so-called active galactic nuclei) appears to be critical in obtaining realistic disk galaxies, to reduce star formation to the slow observed rates and to move gas and metals out of galaxies into the intergalactic medium. This process still has the caveat that “feedback" is modeled phenomenologically and must be tuned to reproduce observed global relations, substantially weakening the predictive power of hydrodynamic simulations.
Cosmic rays consist of charged elementary particles that move incredibly fast, close to the speed of light. Just now, one century after the discovery of cosmic rays, we are starting to understand the astrophysical mechanisms underlying the acceleration of ordinary elementary particles to these enormous velocities: powerful shock waves either driven by exploding stars or by the effects of accretion onto supermassive black holes. Are these cosmic rays just an astrophysical curiosity or do they play a decisive role in the evolution of cosmic structures?
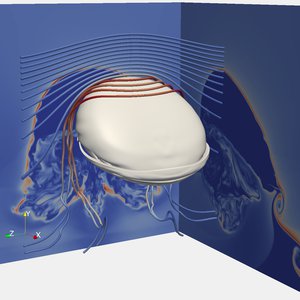
Magnetic draping in a high-resolution magneto-hydrodynamiocs simulation. As the galaxy moves upwards through a weakly magnetized medium a sheath of strong magnetic field is draped around it.
Credit: Pfrommer & Dursi 2010This is one of the central questions that our research would like to answer. First indications point to the possibility that cosmic rays could be responsible for driving powerful gaseous outflows during the formation of galaxies; thus explaining the low star formation observed and solving a major problem of galaxy formation. We are currently working on extending the implementation of cosmic ray physics in the Arepo code to follow the momentum spectrum of hadrons and leptons; we face a challenging five-dimensional problem. Solving this problem enables us to more faithfully follow non-adiabatic cosmic ray cooling, active cosmic ray transport, and non-thermal radiative signatures at radio and gamma-ray energies. We will carry out galaxy formation simulations in an idealized and cosmological framework that self-consistently follow cosmic ray physics and magneto-hydrodynamics. We study the emergence of galactic magnetism that grows via small-scale turbulent and large-scale galactic dynamo processes. We also explore the properties and launching mechanisms of cosmic ray-driven galactic winds, their ability to regulate star formation and to enrich the circumgalactic medium with metals and magnetic fields.
This project (and the three following ones) are funded by the European Research Council (ERC) within the European Union's Framework Program for Research and Innovation, Horizon 2020. Initially, funding was secured through the ERC Consolidator Grant "CRAGSMAN: The Impact of Cosmic Rays on Galaxy and Cluster Formation" (2016-2021 ). The successful new research area will now be continued with the ERC Advanced Grant "PICOGAL: Mind the Gap: from Plasma Kinetics to Cosmological Galaxy Formation" (2022-2027).
Lead investigators: Christoph Pfrommer (PI), Timon Thomas, Joseph Whittingham, Matthias Weber, Larissa Tevlin (all AIP), Philipp Girichidis (Heidelberg), Maria Werhahn, Rüdiger Pakmor and Volker Springel (MPA)
3. Formation and evolution of galaxy clusters
In the course of cosmic evolution, galaxy-sized halos merge with each other to form larger and larger objects, which constitutes the hierarchical growth of cosmic structure. Eventually, galaxy halos merge to form groups of galaxies which themselves merge, culminating in the largest gravitationally bound objects in the Universe: galaxy clusters. Forming at sites of constructive interference of long waves in the primordial density fluctuations, galaxy clusters can be found at the knots of the cosmic web. The gravitational binding energy of galaxy clusters attracts galaxy groups and gas in the cluster vicinity, which accrete and - upon colliding with the cluster gas - form enormous shock waves at cluster boundaries that heat the gas to X-ray emitting temperatures of T ~ 108 K. Rare clusters mergers are the most energetic events in the Universe (after the Big Bang). The accelerated expansion of the Universe, caused by dark energy, delays and eventually stops structure formation. Hence, galaxy clusters sit atop the cosmic mass hierarchy and will remain forever the largest bound objects in our Universe!
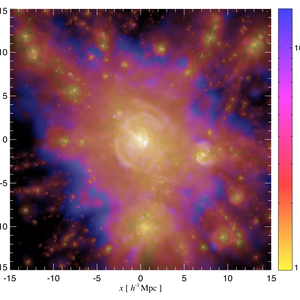
Energy dissipation by cosmic shock waves around a massive galaxy cluster. The brightness scales (logarithmically) with the dissipation rate and the colors indicate the shock strength using Mach numbers
Credit: Pfrommer et al. 2008Similar to galaxy formation, cosmic rays can also play a critical role during the evolution of galaxy clusters, which are an important touchstone in understanding structure formation in our Universe. However, the thermal history of clusters remain mysterious; some central regions should have long since cooled and collapsed, which constitutes the famous "cooling flow problem". Instead, the most massive black holes in the Universe, which are situated at the center of these galaxy clusters, appear to heat the cooling gas at just the right rate. But how does this mechanism work? Again, elusive cosmic rays could come to the rescue and provide the necessary stable heating mechanism. To this end, we are advancing the state-of-the-art in modeling active galactic nuclei feedback by explicitly modeling jets composed of cosmic rays and magnetic fields, aiming at solving the cooling flow problem. We will undertake the challenge to improve our understanding of physical processes leading to the cluster bimodality of cool-core and non cool-core clusters.
Generally, galaxy clusters provide the opportunity to study an "ecosystem" - a volume that is a high-density microcosm of the rest of the Universe. Clusters are signposts for early structure formation and excellent laboratories for studying cosmology, structure formation, and high-energy astrophysics. Current interesting questions include, e.g., how do non-equilibrium processes such as cosmic rays, magnetic fields, and turbulence modify the formation and evolution of galaxy clusters? Do these astrophysical processes jeopardize the use of clusters for cosmological parameter estimation and our quest to understand the dynamical evolution of dark energy with cluster observations? How can galaxy and cluster observations throughout the entire electro-magnetic spectrum from radio to X-ray to gamma-ray wavelengths help in gaining important insight into the underlying plasma physics, high-energy astrophysics, or astroparticle physics, in particular help in elucidating the nature of dark matter? We hope to solve some of these important questions in the upcoming years within our section.
Lead investigators: Christoph Pfrommer, Ewald Puchwein, Joseph Whittingham, Lorenzo Maria Perrone, Léna Jlassi, Rainer Weinberger, Larissa Tevlin, Arne Trabert (all AIP), Thomas Berlok (NBI), Rüdiger Pakmor, Rosie Talbot and Volker Springel (MPA)
4. The interstellar medium and the physics of galactic outflows
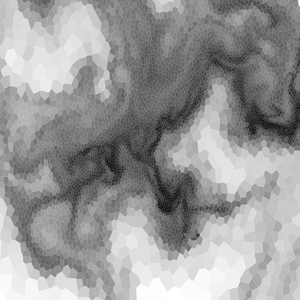
A 1000 degree cold cloudlet (dark colours) moves in a dilute hot wind of one million degrees (white colours). The hot wind deforms the small cloud, leading to the irregular shape seen in the image
Credit: Sparre et al. 2019The space in between the stars is not empty but filled with a diffuse medium out of which new stars are born and to which the products of exploding stars such as heavy metals are delivered once the stars end their lives. This constitutes the interstellar medium, which consist of different phases: from cold and dense molecular clouds that harbour the birthplaces of stars to a hot phase that is created by powerful blast waves of exploding supernovae. Studying the complex interplay of these various phases is not only important for understanding the life cycle of elements but also critical for better understanding galaxy formation since feedback processes impart momentum and energy into the interstellar medium. These processes won't unfold their full potential if the different phases are not modelled as realistically as possible.
A visualization of a simulation of a jellyfish galaxy interacting with the hot magnetized gas in a galaxy cluster. Due to the fast motion of the galaxy, the magnetic field (with the 3 components on the left side) is superimposed on the galaxy (density distribution on the right side) and in the pull of the galaxy the magnetic field lines are aligned at the tail of the galaxy (as seen in the middle magnetic field image). Dense gas can survive if it is transported downstream, because the hot gas in the wind cools down due to the interaction.
Credit: AIP/M. SparreTo this end, we simulate the chemical and dynamical evolution of the multiphase interstellar medium on scales between 1 pc and a few kpc. These Arepo and FLASH simulations (within the SILCC collaboration) use stratified boxes with conditions resembling the solar circle and include magneto-hydrodynamics augmented with cosmic ray physics and self consistent gravity, and follow the thermal evolution using a multi-fluid chemical network with self-shielding. The goal is to understand how supernova-driven supersonic turbulence, cosmic ray pressure and radiative transfer of ultra-violet stellar radiation amplify the magnetic field and self-regulate the interstellar medium. We aim at quantifying the conditions responsible for driving outflows from the disk. In order to move towards more realistic models, which exhibit differential rotation and allow an acceleration of the outflow due to geometric expansion, we plan to simulate the interstellar medium dynamics within a global (dwarf) disk.
These simulations are complemented with idealized super high resolution wind-tunnel Arepo simulations that aim to understand the physics of shattering cooling gas into a cold fog that is embedded in a hot pressurized atmosphere. To do this requires numerically resolving sub-parsec scale droplets of cold gas. We are developing an efficient refinement methodology that allows us to directly simulate the physics of shattering gas in a small volume within a global galaxy formation simulation and to produce mock spectra, which we compare to data. This project has the potential to explain Lyman-alpha halos (observed by MUSE), high velocity clouds in our Milky Way, and the acceleration and entrainment of a cold phase into (cosmic ray driven) galactic outflows.
Lead investigators: Martin Sparre, Timon Thomas, Christoph Pfrommer, Ewald Puchwein, Matthias Weber (all AIP), Philipp Girichidis (Heidelberg)
5. Astroplasma physics: microscopic transport of cosmic rays and heat in galaxies and clusters
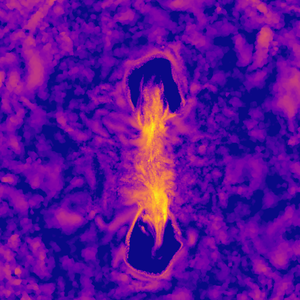
Viscous heating rate in the wake of an AGN jet that results from anisotropic viscosity in magneto hydrodynamics.
Credit: AIP/T. BerlokTo improve our understanding of the macroscopic description of cosmic ray transport, we simulate the microphysics of cosmic ray streaming with kinetic plasma (particle-in-cell) codes. We are developing a coarse-grained hydrodynamic description of cosmic ray streaming and explore the relative importance of the different modes of cosmic ray transport (streaming versus diffusion) for the range of plasma parameters that we encounter in galaxies and clusters. We will validate results of simulations of cosmic-ray propagation in a multiphase medium to observational cosmic ray data.
We plan to perform galaxy cluster simulations with our new implementation of Braginski magneto-hydrodynamics in the Arepo code. This will enable us to scrutinize whether mirror instabilities driven by anisotropic pressure distributions in the collisionless intra-cluster medium are indeed decreasing the transport coefficients of heat and cosmic ray energy density along the magnetic field. In addition, we investigate whether Whistler waves can sustainably modify electron transport in galaxy clusters and thus suppress heat conduction in the thin and hot plasmas of galaxy clusters. This would have far-reaching consequences for thermal instabilities, the propagation of sound waves and magnetic buoyancy instabilities.
Lead investigators: Mohamad Shalaby, Lorenzo Maria Perrone, Virginia Bresci, Rouven Lemmerz, Timon Thomas, Christoph Pfrommer (all AIP), Thomas Berlok (NBI), Jim Drake (U of Maryland), Chris Reynolds (U of Cambridge)
6. Physics and cosmology of blazar heating
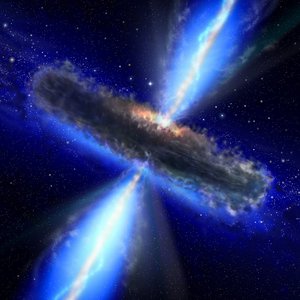
Artist's impression of a super-massive black hole that launches relativistic jets which carry enormous power out to cosmological distances. Blazars are systems where the jet is pointing at us.
Credit: ESA/NASA/AVO-project/PadovaniOne of the last exciting observational frontiers in high-energy astrophysics is the TeV gamma-ray sky. To our surprise, we have recently learned that the extragalactic gamma-ray sky is dominated by "blazars". These are a subclass of super-massive black holes, which are situated at the center of every galaxy and drive powerful relativistic jets and electromagnetic radiation out to cosmological distances. The Universe is opaque to the emitted TeV gamma rays because they annihilate and pair produce on the extragalactic background light emitted by stars in galaxies. The resulting ultrarelativistic pairs of electrons and positrons are commonly assumed to lose energy primarily through inverse Compton scattering with photons of the cosmic microwave background, cascading the original TeV emission a factor of one thousand down to GeV energies.
However, there are two serious problems with this picture. The first is that the expected cascade of GeV emission is not seen in the individual spectra of blazars. Secondly, the emission of all unresolved blazars would overproduce the observed GeV gamma-ray background if these objects share a similar cosmological evolution as the underlying black hole or parent galaxy population. As a putative solution to the first problem, comparably large magnetic fields have been hypothesized which would deflect the pairs out of our line-of-sight to these blazars. However, it would be a challenge to explain these magnetic fields astrophysically, in particular in void regions in between the filamentary cosmic web.
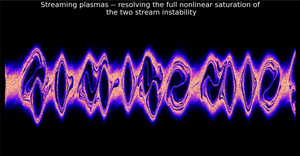
Particle-in-cell simulation of a counter streaming plasma that fully resolves the nonlinear saturation of the two stream instability.
Credit: Shalaby et al. 2017Hence, we have recently found an elegant solution to both problems and argue that there is an even more efficient mechanism competing with this cascading process. Powerful plasma instabilities driven by the highly anisotropic nature of the ultra-relativistic pair distribution provide a plausible way to dissipate the kinetic energy of the TeV pairs locally, heating the intergalactic medium. This naturally solves the two mysteries introduced above in a unified model of blazars and their underlying black hole population without the need to invoke large magnetic fields. Our new model matches the number evolution of these objects observed by the Fermi gamma-ray space telescope as well as the extragalactic gamma-ray background above GeV energies extremely well.
This provides a novel heating mechanism of the gas that fills the space in between galaxies, with a number of important implications for cosmological structure formation. (1) At late times, "blazar heating" deposits more energy per baryon in cosmic voids. This unique property in combination with the increased temperatures is needed to solve many problems present in previous numerical simulations of Lyman-alpha forest spectra. (2) The additional heat increases the pressure of the intergalactic gas at late times, which withstands the gravitational collapse of gas and prevents the late formation of dwarf galaxies. This may partially help resolve the "missing satellites problem" (namely the fact that fewer dwarf satellite galaxies are observed around the Milky Way then predicted by cold dark matter simulations) and may bring the observed early star formation histories into agreement with galaxy formation models. At the same time, it could explain the "void phenomenon" by suppressing the formation of galaxies within existing dwarf halos, thus reconciling the number of dwarfs in low-density regions in simulations and the paucity of those in observations.
Lead investigators: Mohamad Shalaby, Christoph Pfrommer, Ewald Puchwein (AIP), Avery Broderick (Perimeter/U of Waterloo), Phil Chang (UW Milwaukee), Astrid Lamberts (Nice).
7. Testing the Lambda-Cold Dark Matter cosmological model
The simplest cosmological model that seems (largely) consistent with observational constraints is the Lambda-Cold Dark Matter (LCDM) model. In this model, the Universe is filled, in addition to ordinary matter, with dark matter particles that have a negligible initial velocity dispersion, and gravity is described by Einstein's field equations, but including a cosmological constant that drives the observed accelerated expansion of the Universe.
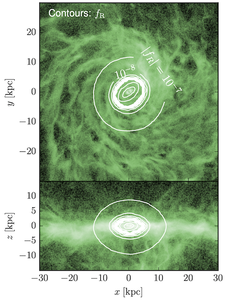
Scalar field (contours) in a disk galaxy in an f(R) gravity model.
Credit: Naik et al. 2018In our section, we test various potential extensions of this basic cosmological model, which may or may not turn out to be needed for matching current and upcoming observational data sets. This includes studies of the free streaming of dark matter particles. In particular, a larger initial velocity dispersion of dark matter particles would suppress their collapse into small structures and would thereby alter cosmic structure growth. Among other things, this would change the density distribution of intergalactic gas that can be probed by observing its absorption properties. We do this by comparing simulations of the intergalactic medium to observations of the forest of Lyman-alpha absorption lines that intergalactic neutral hydrogen imprints on the spectra of distant quasars. Performing such a comparison for simulations based on different dark matter models, e.g., cold and warm dark matter scenarios, allows us to constrain the initial dark matter velocity dispersion, which for a given particle model of dark matter is closely related to the dark matter particle mass.
We also investigate potential deviations of the laws of gravity from general relativity theory. Currently, the best observational constraints on gravity are based on observations within our Solar System, while gravity is tested to much lower accuracy on the scales of galaxies and beyond. We perform cosmological simulations of modified gravity models, e.g., f(R) gravity, to investigate what observational signatures can be used to most sensitively constrain potential deviations from general relativity theory. We then confront our simulations with appropriate observational data sets to put either upper limits on such deviations or to detect them if indeed present.
Lead investigators: Ewald Puchwein (AIP), Noam Libeskind (AIP), Aneesh Naik (RO Edinburgh)
8. Mapping particle physics to cosmology with an effective theory of structure formation - ETHOS
Dark matter is a radical concept that introduces new physics beyond the standard model of particle physics. Following Occam's razor, the emerging standard model of cosmology favors the "cold dark matter scenario", which postulates a new elementary particle that interacts with baryonic matter via gravitation only and requires no other fundamental interaction. On large cosmological scales, this empirical model has been able to successfully explain all different cosmological observations and tests. However, on small scales there are a number of puzzling results that may or may not be fully explained within this model. This is because the standard model of cosmology is not predictive on these scales due to the very complex gas-dynamical processes that are mainly responsible for the luminosity of galaxies. How can we test this cold dark matter paradigm? While the standard model of particle physics requires 3 fundamental forces (other than gravity) would it be natural to assume that there is no new fundamental interaction in the dark sector? If not, what experiment could we design to probe and constrain such a hypothetical new dark-sector interaction?

Interaction processes that set the dark matter relic density and may lead to observable neutrinos today (left), change the inner density of dwarf halos (middle) and induce a large cutoff in the spectrum of primordial density perturbations (right).
Credit: van den Aarssen et al. 2012To answer these questions, we formulate an Effective THeory Of Structure formation (ETHOS) that enables cosmological structure formation to be computed in almost any micro-physical model of dark matter physics. This framework maps the detailed micro-physical theories of particle dark matter interactions into the physical effective parameters that shape the linear matter power spectrum and the self-interaction transfer cross section of non-relativistic dark matter. These are the input to structure formation simulations, which follow the evolution of the cosmological and galactic dark matter distributions. Models with similar effective parameters in ETHOS but with different dark particle physics would nevertheless result in similar dark matter distributions. Taken together, these effective parameters in ETHOS allow the classification of dark matter theories according to their structure formation properties rather than their intrinsic particle properties, paving the way for future simulations to span the space of viable dark matter physics relevant for structure formation, including galaxy formation (at the faint and bright end), reionization, and the Lyman-alpha forest.
Lead investigators: Francis-Yan Cyr-Racine (Harvard), Jesus Zavala (Reykjavik), Mark Vogelsberger (MIT), Christoph Pfrommer (AIP), Torsten Bringmann (UIO), Kris Sigurdson (UBC), Sownak Bose (Harvard)
9. Cosmological simulations database - CosmoSim
As a service to the community, the CosmoSim database provides results from cosmological simulations performed within different projects. So far it hosts the MultiDark and Bolshoi project, and the CLUES project. In the future, we plan to enter HESTIA simulation data products.
The database can be queried by entering SQL statements directly into the Query Form or via Scripted access. Main research topics are Statistical analysis of large scale structure, Baryonic oscillations and Cosmological models.
Investigators (in alphabetic order): Andrew Benson (Carnegie), Sofia A. Cora, Darren Croton (Swinburne), Harry Enke (AIP), Anastasia Galkin (AIP), Stefan Gottloeber (AIP), Noam Libeskind (AIP), Anatoly Klypin (NMSU), Alexander Knebe (UAM), Kristin Riebe (AIP), Matthias Steinmetz (AIP), Gustavo Yepes (UAM).